QM Basics 4 : Particle Entanglement
<< Rome, Italy,JUN 28 2024 >> << Re Factored DEC 19 2024 >> Quantum Entanglement: A Fundamental Phenomenon Quantum entanglement is a foundational concept in quantum mechanics, describing a situation where two or more particles become correlated in such a way that the state of one particle is directly tied to the state of another, regardless of the physical distance separating them. This means that measuring the state of one particle instantly reveals the state of its entangled partner, even if they are light-years apart. Albert Einstein famously referred to this phenomenon as “spooky action at a distance,” reflecting his skepticism toward the implications of entanglement. Although Einstein contributed significantly to the development of quantum mechanics, he remained uneasy about its counterintuitive nature. At the heart of entanglement lies the principle that quantum particles, such as electrons or photons, do not possess definite states until they are measured. Before measurement, they exist in a superposition of states, meaning they simultaneously embody multiple possibilities. When two particles are entangled, their properties—such as spin or polarization—become interdependent. If the state of one particle is measured, its wavefunction collapses, and the entangled particle’s wavefunction instantaneously collapses into a corresponding state. This interconnection between superposition and entanglement highlights the deeply intertwined nature of quantum phenomena. The peculiar nature of entanglement challenges classical notions of how objects should behave. Classical physics asserts that objects possess definite properties independent of observation and that no influence can travel faster than the speed of light. Entanglement defies these principles, suggesting that particles share information in a manner that transcends space and time. However, this phenomenon does not violate relativity, as the instantaneous correlations between entangled particles cannot be used to transmit information faster than light. Instead, the outcomes of measurements remain random, ensuring that the principles of causality and the speed of light remain intact. Applications and Experimental Validation Quantum entanglement is not merely a theoretical curiosity; it has practical implications in emerging technologies. In quantum computing, entangled qubits enable faster and more efficient processing by simultaneously representing multiple possibilities. In quantum cryptography, entanglement is used to establish secure communication channels that are inherently resistant to eavesdropping. Any attempt to intercept these channels would disturb the entangled state, making tampering detectable. These applications illustrate how entanglement is driving innovation in technology, with implications for fields such as secure communications and computational efficiency. The existence of entanglement has been repeatedly validated through rigorous experimentation, including tests of Bell’s theorem. These experiments have consistently demonstrated that quantum entanglement is real and that its predictions align with quantum mechanics rather than classical physics. While its implications continue to provoke philosophical debate, the practical potential of entanglement is reshaping our understanding of technology and the universe itself. Future discussions will focus on the military applications of these advancements, starting with quantum cryptography. Why Macroscopic Objects Do Not Exhibit Quantum Behavior Macroscopic objects fail to display quantum behavior due to the phenomenon of decoherence, which occurs when quantum systems interact with their environments. In quantum mechanics, particles exist in superpositions of states, but as the size and complexity of a system increase, interactions with the environment grow exponentially. These interactions cause the system's quantum states to "collapse" into classical states, suppressing observable quantum effects. Consequently, macroscopic objects, which are constantly influenced by their surroundings, behave in ways consistent with classical physics. Additionally, quantum phenomena such as tunneling and uncertainty are significant only at extremely small scales, where energies and distances are comparable to Planck’s constant. For larger objects, the associated wavelengths become vanishingly small, rendering wave-like properties undetectable. For instance, while an electron's wave-like behavior is observable, the wavelength of a macroscopic object like a baseball is so small that quantum interference cannot be observed. Lastly, the deterministic behavior of macroscopic systems arises from the collective averaging of vast numbers of particles. The probabilistic nature of quantum mechanics is overshadowed by the predictable, smooth behavior of large-scale systems, aligning them with classical laws such as Newtonian mechanics. This transition from quantum to classical mechanics ensures that the underlying quantum rules remain consistent with our macroscopic reality. Theoretical Interpretations of Quantum Mechanics Several interpretations seek to explain the perplexing behavior of quantum mechanics, each offering unique perspectives on the role of observation, wavefunction collapse, and the nature of reality. The Copenhagen Interpretation Formulated by Niels Bohr and Werner Heisenberg, the Copenhagen interpretation posits that quantum systems exist in superpositions until measured, at which point the wavefunction collapses into a definite state. This interpretation emphasizes the probabilistic nature of quantum mechanics and the active role of the observer. Bohmian Mechanics This deterministic framework introduces "hidden variables" to account for quantum behavior. Particles in Bohmian mechanics are guided by a "pilot wave" that dictates their motion. Unlike the Copenhagen interpretation, it avoids randomness but requires non-locality to explain phenomena like entanglement. The Many-Worlds Interpretation (MWI) Rejecting wavefunction collapse, MWI proposes that all possible outcomes of a quantum measurement occur in separate, parallel universes. While deterministic, this interpretation introduces the counterintuitive notion of countless branching realities. Here at TDM, we favor the Copenhagen interpretation for its intuitive alignment with the probabilistic nature of quantum mechanics and its avoidance of metaphysical complications like infinite parallel universes. Key Features of Quantum Mechanics To summarize, there are four critical principles of quantum mechanics: Particles are not waves, but wave-like behavior is essential to describe them. Certain particle properties—such as energy, charge, and spin—are quantized. Quantum systems exhibit inherent uncertainty. Quantum effects are only observable at extremely small scales. These principles form the foundation of quantum mechanics, guiding its applications in both theoretical and practical contexts. Moving forward, we will explore the practical uses of quantum mechanics, beginning with quantum cryptography in military systems. References Stephen Hawking & Leonard Mlodinow, The Grand Design, Bantam Books,2012 Jakob Schwictenburg, No-Nonsense Quantum Mechanics, No-Nonsense Books,2020
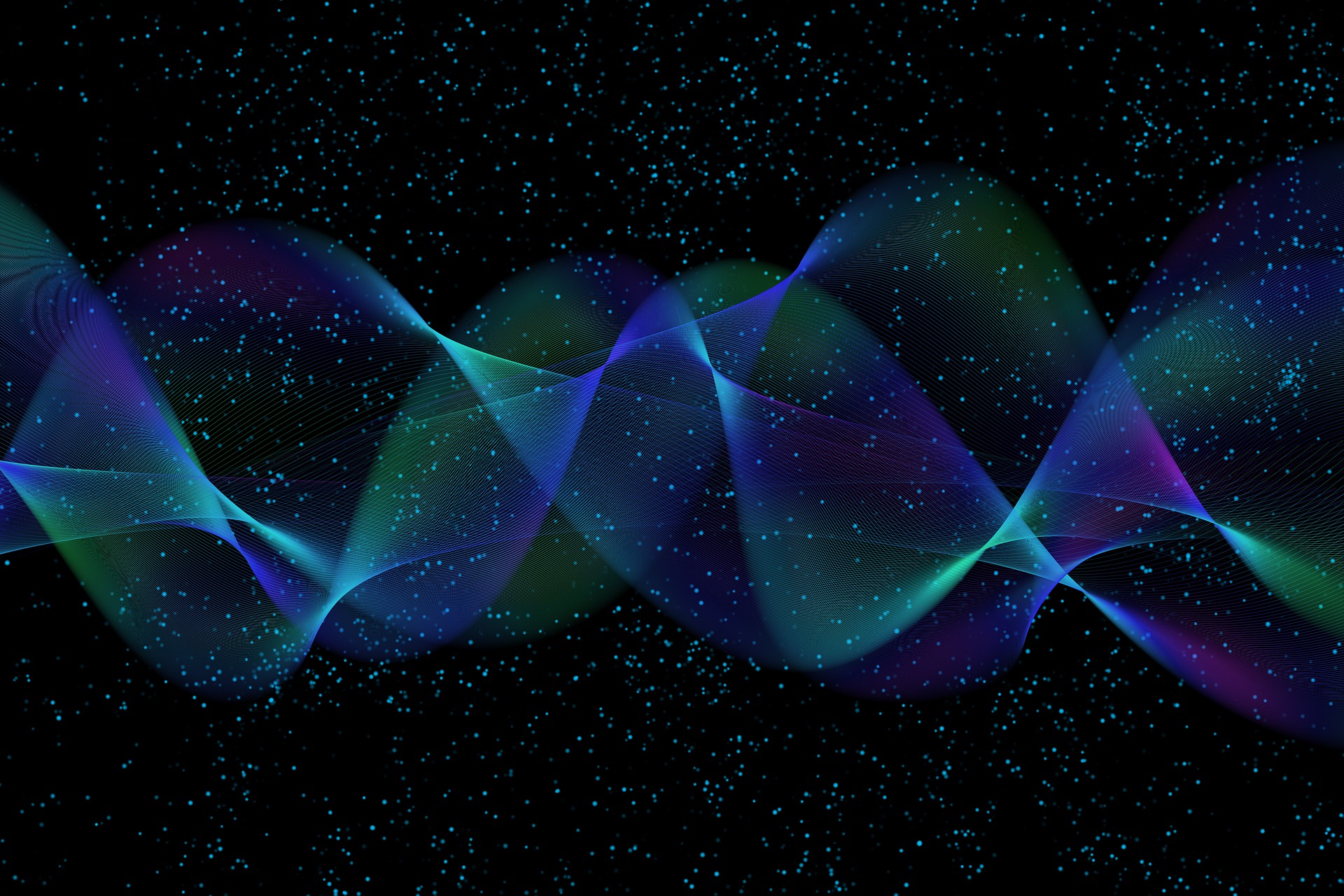